This year is an historic one for nuclear power, with the first reactors winning U.S. government approval for construction since 1978. Some have seen the green lighting of two Westinghouse AP1000 reactors to be built in Georgia as the start of a revival of nuclear power in the West, but this may be a false dawn because of the problems besetting conventional reactors. It may be that when a new boom in nuclear power comes, it won't be led by giant gigawatt installations, but by batteries of small modular reactors (SMRs) with very different principles from those of previous generations. But though a technology of great diversity and potential, many obstacles stand in its path. Gizmag takes an in-depth look at the many forms of SMRs, their advantages, and the challenges they must overcome.
Globally, there is a growing demand for electricity that is cheap, reliable and abundant. There's also an increasing need to find sources of energy that do not rely on doing business with hostile or unstable nations. At the same time, recent concerns over global warming have resulted in many governments pledging their nations to reduce the amount of carbon dioxide they generate and new, stricter environmental regulations threaten to close coal-powered plants across Europe and the United States. The hope was that massive investments in alternative technologies such as solar and wind power would make up for this cut in generating capacity, but the inefficiencies and intermittent nature of these technologies made it clear that something with the capacity and reliability of coal and natural gas plants was needed. Nuclear, in other words.
The problem is that nuclear energy is the proverbial political hot potato - even in early days when the new energy source exploded onto the world scene. The tremendous amount of energy locked in the atom held the promise of a future like something out of a technological Arabian Nights. It would be a world where electricity was too cheap to meter, deserts would bloom, ships would circle the Earth on a lump of fuel the size of a baseball, planes would fly for months without landing, the sick would be healed and even cars would be atom powered. But though nuclear power did bring about incredible changes in our world, in its primary role, generating electricity for homes and industry, it ended up as less of a miracle and more of a very complicated way of boiling water.
Not only complicated, but expensive and potentially dangerous. Though hundreds of reactors were built all over the world and some countries, such as France, generate most of their electricity from it, nuclear power has faced continuing questions over cost, safety, waste disposal and proliferation. One hundred and four nuclear plants provide the United States with 20 percent of the nation's power, but a building permit hadn't been issued since 1978 with no new reactors coming on line since 1996 and after the uproar from the environmental movement after nuclear accidents at Three Mile Island, Chernobyl and Fukushima, it seemed unlikely that any more would ever be approved - until now. This fierce domestic opposition to nuclear power has caused many governments to take an almost schizophrenic stance regarding the atom.
Germany, for example, decided to abandon nuclear power completely in favor of alternative energy, but then the severe winter of 2011-12 got so cold that the Danube was freezing and Berlin had to put some of the mothballed reactors back into service. This opposition also means that many Western countries have a shortage of nuclear engineers because many see it as a dying industry not worth getting into. This is particularly acute in the United States and Britain, neither of which have retained the capacity for building the huge reactor vessels and must farm this out to overseas manufacturers.
Worse, nuclear power suffers from the natural gas boom brought on by new drilling techniques and fracking that opened up vast new gas fields in the West and dropped the price of gas to the point where coal and nuclear have a hard time matching it.
And money is one of the key problems facing a revival of nuclear power. Up until now, the sort of reactors used for generating electricity have tended toward the gigantic with reactors reaching gigawatt levels of output. With plants that large, small wonder that the cost of construction combined with obtaining permits, securing insurance and meeting legal challenges from environmentalist groups can push the cost of a conventional nuclear plant toward as much as US$9 billion. It also means very long build times of ten or fifteen years. This isn't helped by the fact that nuclear plants are custom designed from scratch in multi-billion dollar exercises in re-inventing the wheel. With so much time and money involved, an unforeseen change in regulations or discovery of something like a geological fault under the reactor site can make this a case of putting a lot of very expensive eggs in a very insecure basket.
Then there are safety issues. Reactor design is safer today than ever before. The Fukushima accident happened because Fukushima's reactors are a very old design - as old as the oldest active American reactors. If the earthquake and tsunami that hit Fukushima had hit a modern reactor, the disaster probably would never have happened. However, large conventional reactors still have safety issues because they require very fast reaction times to prevent damage in the event of an accident. Accidents can progress so fast in a reactor that the operators must take action within hours, perhaps even minutes. If a meltdown accident does occur, the large amount of fuel in the reactor means that a great deal of radioactive material may be released into the atmosphere. That makes time an essential element.
The enriched uranium fuel used in conventional reactions also poses a problem for nuclear weapons proliferation. Contrary to popular belief, the uranium used in reactors and even the plutonium that some reactors produce are useless for building nuclear bombs (the isotope ratios are all wrong), but the processes needed to produce nuclear fuel and bomb materials are almost exactly the same. So, though conventional reactors may not be a proliferation threat, the enrichment plants that service them are.
Small Modular Reactors
One way of getting around many of these problems is through the development of small modular reactors (SMR). These are reactors capable of generating about 300 megawatts of power or less, which is enough to run 45,000 US homes. Though small, SMRs are proper reactors. They are quite different from the radio-thermal generators (RTG) used in spacecraft and remote lighthouses in Siberia. Nuclear reactors such as SMRs use controlled nuclear fission to generate power while RTGs use natural radioactive decay to power a relatively simple thermoelectric generator that can only produce, at most, about two kilowatts.In terms of power, RTGs are the equivalent of batteries while small nuclear reactors are only "small" when compared to conventional reactors. They are hardly the sort that you would keep in the garage. In reality, SMR power plants would cover the area of a small shopping mall. Still, such an installation is not very large as power plants go and a reactor that only produces 300 megawatts may not seem worth the investment, but the US Department of Energy is offering US$452 million in matching grants to develop SMRs and private investors like the Bill Gates Foundation and the company of Babcock and Wilcox are putting up money for their own modular reactor projects.
The 60-year old breakthrough
One reason for government and private industry to take an interest in SMRs is that they've been successfully employed for much longer than most people realize. In fact, hundreds have been steaming around the world inside the hulls of nuclear submarines and other warships for sixty years. They've also been used in merchant ships, icebreakers and as research and medical isotope reactors at universities. There was even one installed in the Antarctic at McMurdo Station from 1962 to 1972. Now they're being considered for domestic use.
The case for SMRs
SMRs have a number of advantages over conventional reactors. For one thing, SMRs are cheaper to construct and run. This makes them very attractive to poorer, energy-starved countries; small, growing communities that don't require a full-scale plant; and remote locations such as mines or desalination plants. Part of the reason for this is simply that the reactors are smaller. Another is that, not needing to be custom designed in each case, the reactors can be standardized and some types built in factories that are able to employ economies of scale. The factory-built aspect is also important because a factory is more efficient than on-site construction by as much as eight to one in terms of building time. Factory construction also allows SMRs to be built, delivered to the site, and then returned to the factory for dismantling at the end of their service lives - eliminating a major problem with old conventional reactors, i.e. how to dispose of them.SMRs also enjoy a good deal of design flexibility. Conventional reactors are usually cooled by water - a great deal of water - which means that the reactors need to be situated near rivers or coastlines. SMRs, on the other hand, can be cooled by air, gas, low-melting point metals or salt. This means that SMRs can be placed in remote, inland areas where it isn't possible to site conventional reactors.
Safety
This cooling system is often passive. In other words, it relies more on the natural circulation of the cooling medium within the reactor's containment flask than on pumps. This passive cooling is one of the ways that SMRs can improve safety. Because modular reactors are smaller than conventional ones, they contain less fuel. This means that there's less of a mass to be affected if an accident occurs. If one does happen, there's less radioactive material that can be released into the environment and makes it easier to design emergency systems. Since they are smaller and use less fuel, they are easier to cool effectively, which greatly reduces the likelihood of a catastrophic accident or meltdown in the first place.This also means that accidents proceed much slower in modular reactors than in conventional ones. Where the latter need accident responses in a matter of hours or minutes, SMRs can be responded to in hours or days, which reduces the chances of an accident resulting in major damage to the reactor elements.
The SMR designs that reject water cooling in favor of gas, metal or salt have their own safety advantages. Unlike water-cooled reactors, these media operate at a lower pressure. One of the hazards of water cooling is that a cracked pipe or a damaged seal can blow radioactive gases out like anti-freeze out of an overheated car radiator. With low-pressure media, there's less force to push gases out and there's less stress placed on the containment vessel. It also eliminates one of the frightening episodes of the Fukushima accident where the water in the vessel broke down into hydrogen and oxygen and then exploded.
Another advantage of modular design is that some SMRs are small enough to be installed below ground. That is cheaper, faster to construct and less invasive than building a reinforced concrete containment dome. There is also the point that putting a reactor in the ground makes it less vulnerable to earthquakes. Underground installations make modular reactors easier to secure and install in a much smaller footprint. This makes SMRs particularly attractive to military customers who need to build power plants for bases quickly. Underground installation also enhances security with fewer sophisticated systems needed, which also helps bring down costs.
SMRs can help with proliferation, nuclear waste and fuel supply issues because, while some modular reactors are based on conventional pressurized water reactors and burn enhanced uranium, others use less conventional fuels. Some, for example, can generate power from what is now regarded as "waste", burning depleted uranium and plutonium left over from conventional reactors. Depleted uranium is basically U-238 from which the fissible U-235 has been consumed. It's also much more abundant in nature than U-235, which has the potential of providing the world with energy for thousands of years. Other reactor designs don't even use uranium. Instead, they use thorium. This fuel is also incredibly abundant, is easy to process for use as fuel and has the added bonus of being utterly useless for making weapons, so it can provide power even to areas where security concerns have been raised.
But there's still the sticking point that modular reactors are, by definition, small. That may be fine for a submarine or the South Pole, but what about places that need more? Is the alternative conventional nuclear plants? It turns out that the answer is no. Modular reactors don't need to be used singly. They can be set up in batteries of five or six or even more, providing as much power as an area needs. And if one unit needs to be taken off line for repairs or even replacement, it needn't interfere with the operation of the others.
Types of modular reactors
Let's take a look now at some of the major types of modular reactors under development. There are, in fact, many more than are presented here, but this should give a good cross section of what is in the pipeline.
Light-water reactors
A modular light-water reactor is basically a scaled-down version of a conventional reactor. Like conventional reactors, it uses water as a coolant and a neutron moderator (that is, the water slows down the neutrons produced by the nuclear fuel so that the uranium atoms have a better chance of absorbing them and inducing nuclear fission. The trick of fission is simply to have enough nuclear fuel in one place with a moderator so that the reaction becomes self-sustaining). Engineers already have decades of experience with light-water SMRs because these are the type used on submarines and icebreakers, so the technology is already advanced and has had lots of field testing under very hard conditions. Imagine a nuclear power plant that has to be able to operate safely as it's being tossed about in the ocean while sealed inside a submarine hull and you can see the daunting challenges that have been overcome.Small light-water reactors aren't as efficient as their larger cousins, but they have a number of advantages. Steam is produced in a nuclear plant by passing a loop of cooling water from the reactor through the steam generator, which is a separate vessel filled with coiling pipes. The hot cooling water enters the generator and as it runs through the pipes a second coil filled with water is heated by the water from the reactor. This changes to steam, which turns the turbines that turns the dynamos. On a conventional reactor, most types have the steam generator outside the reactor vessel. With light-water SMRs, the steam generator can be placed inside the vessel. This not only makes the reactor more compact and self-contained, but it also makes it much safer. One common problem in reactors is radioactive water leaking as it travels from the reactor to the steam generator. With the steam generator inside the reactor vessel, it's the much safer situation of only non-radioactive water/steam going into and out of the reactor vessel.
Westinghouse SMR
The Westinghouse SMR is a miniature version of their AP1000 reactor. But where the AP1000 produces 1,154 megawatts and requires a plant covering 50 acres (20 ha), the Westinghouse SMR needs only 15 (6 ha), puts out 225 megawatts and can be built in 18 months as opposed to several years. The reactor and containment vessel stand 89 feet (27 m) high and 32 feet (9.8 m) in diameter, which makes it compact enough to be factory-built and shipped by rail to the site. Its fuel is standard enriched uranium that needs servicing every two years, but the reactor's passive cooling system relies on the natural circulation of water rather than pumps, which means that even in the event of a complete power loss, as Fukushima suffered, the Westinghouse SMR can go for up to a week without needing any operator intervention to prevent damage.
mPower
Backed by Babcock and Wilcox, mPower is based on US Navy reactor designs and produces 160 megawatts when the system's condensers are cooled by water, but it can be air-cooled as well, though with a lower power output. Seventy-five feet (23 m) high and 14 feet (4.3 m) in diameter, mPower is designed to be factory built, rail-shipped and installed below ground. Like the Westinghouse SMR, the mPower uses a passive cooling system and the steam generator is integral with the reactor. Unlike the Westinghouse SMR, the mPower needs refueling only every four years and the process involves simply replacing the entire core, which is inserted like a cartridge. The reactor has a 60-year service life and is designed to store its spent fuel on site for the duration.
NuScale
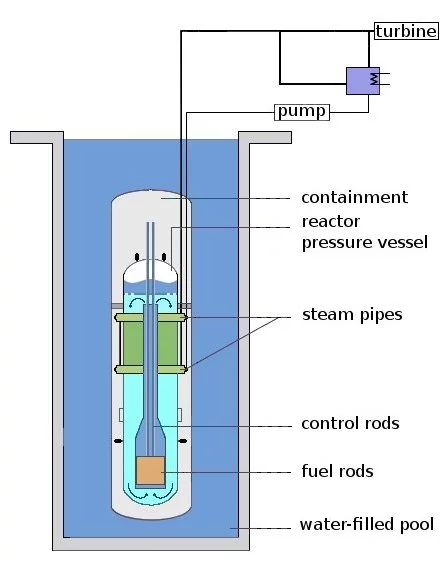
NuScale seems impractically small with its output of only 45 megawatts, but it's intended to be installed twelve at a time to provide up to 540 megawatts. These are each placed in an underground pool of water and each unit is cooled by natural circulation. Because of this, there are no pumps and the only moving parts in the reactor are those used to operate the control rods. When it is time for refueling, the reactor is removed from its pool by an overhead crane and taken to another section of the facility.
High-temperature gas cooled reactors
As the term implies, gas-cooled reactors use a gas instead of water as a reactor cooling medium. In modern reactors this gas is usually helium because it's an inert element that doesn't react with other materials, yet is an excellent coolant (just ask any mixed-gas deep sea diver and he'll tell you why they have a heating tube in their suit while breathing helium). This is important because, not using water, the moderator for the nuclear reaction is a graphite core, which is flammable. These operate at relatively low pressures and high gas temperatures of up to 1,800 degrees F (1,000 degrees C) and the gas either drives the turbines directly or via a steam generator. This reactor type has safety advantages because the way the design makes the nuclear reaction self-regulating. As the reactor gets hotter, the reaction slows down and the reactor cools. It also lends itself to smaller scales to allow for factory building and underground installation.
GT-MHR
Built by a partnership led by General Atomics, the GT-MHR reactor has a capacity of 285 megawatts and can also be used to produce 100,000 tons of hydrogen gas per year. It has the interesting distinction of being able to run on weapons-grade plutonium. The reason for this was that the GT-MHR was originally designed to help dispose of Soviet nuclear warheads after the end of the Cold War. It also serves to highlight the practical applications of the SMRs' ability to burn alternative nuclear fuels.
Fast neutron reactors
In conventional reactors, neutrons are slowed down by a moderator such as water, carbon or helium so that the uranium atoms have a better chance of absorbing them and initiating fission. A fast neutron reactor manages the same fission reaction except it does so by reflecting fast-moving neutrons back into the uranium in large quantities and thereby increasing the odds of fission. This has the advantage of allowing reactors to be very simple in design (and hence smaller) and to use enriched fuels, thorium or even nuclear waste as fuel.There are two types of fast neutron systems used in current SMR designs. The first are candle, breed-burn or traveling-wave reactors. The second, standing wave reactors.
The "candle" name for the first variety stems from the fact that that's what the fuel resembles. Put simply, it's a big slab of depleted uranium with a plug of enriched uranium stuck in one end. When the nuclear reaction starts, the enriched uranium "ignites" the slab by initiating a reaction that turns the U-238 into Pu-239, an isotope of plutonium that can fission and generate power. This reaction burns along the slab at roughly one centimeter per year, creating and burning plutonium as it goes. It's a process that can take years, even decades, as the reactor burbles away at a temperature of about 1,000 degrees F (550 degrees C) while cooled by liquid sodium, lead or lead-bismuth alloy.
The other version is called a "standing wave," and the principle is the same, except instead of a great slab, the reactor is made up of fuel rods of U-238 and the reaction is started in the center. As the reaction proceeds outwards, the spent rods are reshuffled by the operators until all the fuel is consumed. The upshot of this is that a traveling wave reactor uses it fuel more efficiently and can run for 60 years without refueling. Theoretically, it could go for 200 years.
With either type, they are also unusual in that they have no moderator, rely on passive cooling, can be built in factories and have no moving parts. They are as close to plug-and-play as nuclear reactors can get.
Hyperion
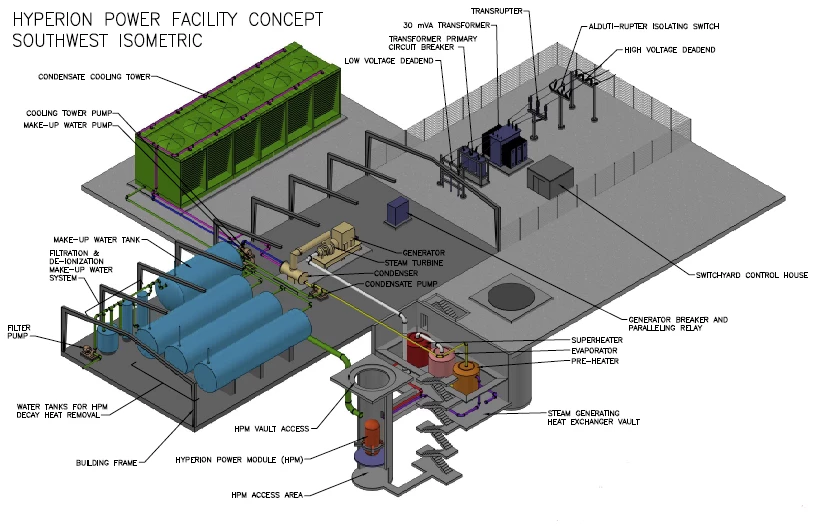
Hyperion is another very small modular reactor that produces only 25 megawatts, but what it lacks in power it makes up for in portability. The reactor vessel is only 8 feet (2.5 m) tall and 5 feet (1.5 m) in diameter, has no moving parts and can go for ten years without refueling. When refueling is needed, the reactor is returned to the factory and replaced rather in the manner of a gas bottle. This configuration not only makes it possible to build multi-reactor power plants, but the individual reactors can also be used for applications like providing heat to extract oil from shale beds, steam for industrial uses and running desalination plants.
PRISM
Power Reactor Innovative Small Module (PRISM) is a GE-Hitachi design. It's sodium cooled, installed underground and generates 311 megawatts with refueling every six years. Its ability to burn plutonium and depleted uranium makes it of great interest to the UK, which is negotiating to have two installed at the Sellafield nuclear facility where they would be used to burn nuclear waste stockpiles. This is more than just a waste disposal solution. It's estimated that if this works, the waste could provide power to Britain for 500 years.
Molten salt reactors
In this type of SMR, the coolant and the fuel are one in the same. The coolant is a mixture of lithium and beryllium fluoride salts. In this is dissolved a fuel, which can be enriched uranium, thorium or U-233. This molten salt solution passes at relatively low pressure and a temperature of 1,300 degrees F (700 degrees C) through a graphite moderator core. As the fuel burns, the waste products are removed from the solution and fresh fuel is added.
Flibe
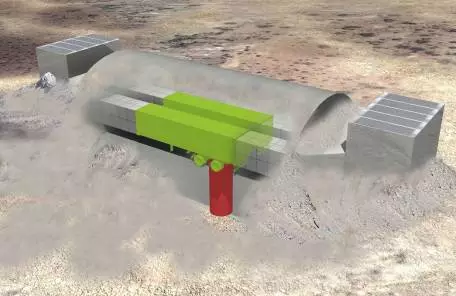
Flibe (Fluoride salt of Lithium and Beryllium) is a sort of reactor in a box. The US military wants to develop small reactors that can be easily set up at remote bases. Toward this end, the Flibe is designed around a power plant that packs into a set of cargo containers. The idea is to stick the reactor in the ground, set up the generating machinery and cover the lot with a building. The last doesn't need to be anything like the containment building of a conventional reactor because the reactor is not only passively heated, but also features a salt plug that needs to be actively cooled at all times. If the reactor suffers a breakdown and the reactor starts to overheat, the plug melts and the molten salt/fuel mixture pours out into a drain tank. Power output is rated at 20 to 50 megawatts and it uses U-233 and thorium for fuel. This not only eliminates proliferation issues (neither U-233 nor thorium is completely unsuitable for weapons), but it also opens up a cheap, easily obtained energy source.
Challenges remain
As impressive as many of these reactors sound, most of them are still in one stage or another of development or approval. It is a long way from there to flipping a switch and watching the lights go on. Most of these designs have roots that go back over half a century.In the 1950s, Admiral Hyman Rickover, the architect of the US nuclear fleet, pointed out that the small research reactors, the precursors of SMRs, had a lot of advantages. They were simple, small, cheap, lightweight, easy to build, very flexible in design and needed very little development. On the other hand, practical reactors must be built on schedule, need a huge amount of development spent on "apparently trivial matters", are expensive, large, heavy and complicated. In other words, there's a large gap between what is promised by a technology in the design phase and what it ends up as once it's built.
So it is with the current stable of SMRs. Many hold great promise, but they have yet to prove themselves. Also, they raise many questions. Will an SMR need fewer people to run it? What are its safety parameters? Will they fulfill current regulations? Will the regulations need to be changed to suit the nature of SMRs? Will evacuation zones, insurance coverage or security standards need to be altered? What about regulations regarding earthquakes?
Indeed, it is in government regulations that the modular reactors face their greatest challenges. Whatever the facts about nuclear accidents from Windscale to Fukushima, a large fraction of the public, especially in the West, is very nervous about nuclear energy in any form. There are powerful lobbies opposed to any nuclear reactors operating and the regulations written up by governments reflect these circumstances. Much of the cost of building nuclear plants is due to meeting all regulations, providing safety and security systems, and just dealing with all the legal barriers and paperwork that can take years and millions of dollars to overcome. Modular reactors have the advantage of being built quickly and cheaply, which makes them less of a financial risk, and factory manufacturing means that a reactor intended for a plant that missed approval can be sold to another customer elsewhere. And some SMRs are similar enough to conventional reactors that they don't face the burden of being a "new" technology under skeptical scrutiny. However, red tape is still a very real thing.
Only time will tell if the small reactor becomes a common sight on our power grids, if it falls by the wayside like other technological dreams, or if it falls victim to the bureaucrats' rule book.