Batteries. We buy them at the store, use them up, and throw them away without much thought. In reality, however, batteries are remarkably complex electrochemical devices that are continually evolving. The latest example of this comes from the Lawrence Berkeley National Laboratory, where researchers have invented an advanced lithium/sulfur (Li/S) cell that offers a unique combination of energy storage, power, recharge speed, and survivability.
Lithium/sulfur rechargeable batteries offer a remarkably large capacity for energy storage, mainly because two electrons are produced each time a molecule is processed through the battery's chemistry.
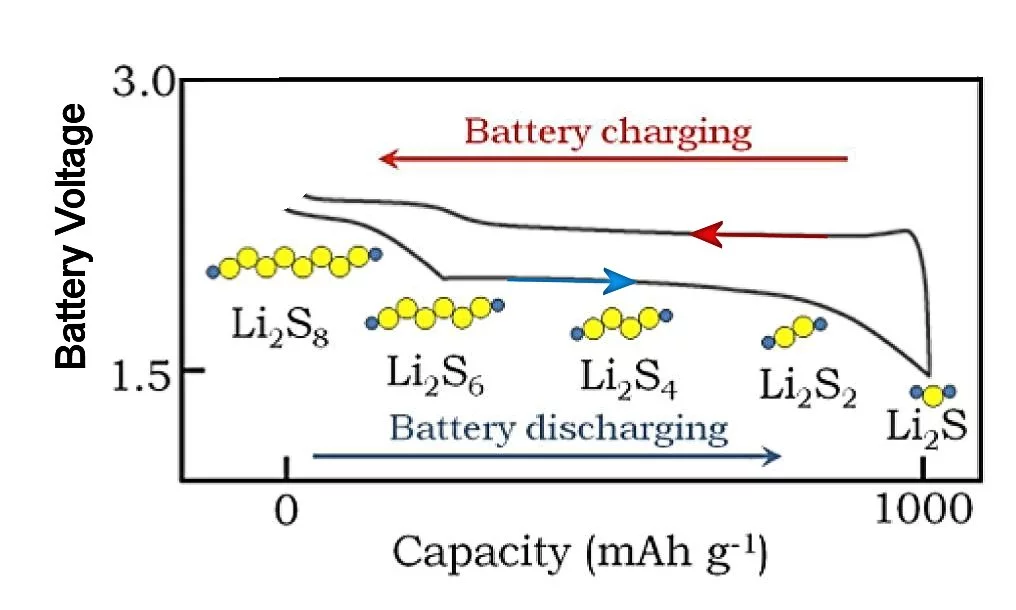
A basic Li/S cell consists of a lithium anode, a carbon-sulfur cathode, and an electrolyte that permits lithium ions to pass. The overall cell reaction during discharge converts lithium metal in the anode into Li2S at the surface of the cathode. The flow of two lithium ions from the anode to the cathode is then balanced by the flow of two electrons between the battery contacts, delivering double the current of a Li-ion battery at a voltage between about 1.7 and 2.5 volts, depending on the state of charge of the cell. Lithium polysulfides are formed at intermediate charge levels, which affect the cell voltage as indicated above.
That's the good news. The bad news involves a host of materials problems associated with the basic Li/S chemistry and some side reactions. When the sulfur in the cathode absorbs lithium ions from the electrolyte, the Li2S has nearly double the volume of the original sulfur. This is a very large source of mechanical stress on the cathode, which causes mechanical deterioration, reduces the electrical contact between the carbon and the sulfur (the path whereby electrons flow to allow the reaction to occur), and prevents the flow of lithium ions to the sulfur surface.
Another problem is that lithium and sulfur generally don't react immediately to form Li2S, but rather get there through a series of intermediate species, such as Li2S8, Li2S6, etc. Sulfur itself and Li2S are essentially insoluble in the typical electrolyte used in Li/S cells, but these intermediate "polysulfides" often are soluble, which causes an ongoing and severe loss of sulfur at the cathode. Other problems appear, such as a roughening of the lithium anode surface with large charge or discharge currents. All of these problems result in a basic Li/S cell being a very bad battery.
The Li/S battery chemistry, however, offers the potential for such wonderful battery performance that, since its discovery in the 1960s, a lot of work has been aimed at solving these problems. Engineers and scientists have tried putting the sulfur inside nanochannels as well as using lithium-silicon-carbon alloy anodes, sulfur polymer cathodes, and a host of other imaginative attempts at solving the interlocked Li/S battery performance limitations. While a good deal of progress has been made, development of a practical Li/S cell has eluded researchers for half a century.
The Lawrence Berkeley team addressed these problems by developing a nanocomposite cathode that addresses the three main problems presented by Li/S cells. The new cathode material is a sulfur-graphene oxide nanocomposite held together using an elastic polymer binder.
Graphene oxide is formed from graphite oxide by exfoliation, in which an ultrasonic field is applied to graphite oxide while suspended in water. The ultrasonic waves peel apart the layers of the graphite oxide, producing very thin flakes of graphene oxide.
These flakes are then given a coating of sulfur a few nanometers in thickness. The thinness of the sulfur coating allows the sulfur atoms to make good electrical contact with the graphene oxide flakes. While not an excellent conductor of electricity, graphene oxide has sufficient conductivity to anchor the sulfur to the cathode, thereby permitting a large flow of current to pass through the sulfur layers.
There are intermediate products (lithium polysulfides) resulting from the operation of a Li/S cell that can dissolve into the ionic electrolyte of the cell, thereby causing sulfur loss and degrading the cell's storage capacity. One effect of putting the sulfur on the graphene oxide nanoflakes is to protect one side of the sulfur layer against this degradation.
In the new Li/S cell, a protective surfactant is placed atop the sulfur layer to also protect its surface against dissolving in the electrolyte. Because the surfactant is cationic (attracted to the sulfur), it will let the lithium anions through to react with the sulfur of the cathode while protecting the sulfur layer. As any lithium polysulfides formed under the surfactant are trapped there, this addition nearly eliminates the problem of sulfur loss.
To form a useful cathode for an Li/S cell, this loose collection of coated graphene oxide nanoflakes must be bound together to form a nanocomposite with a very large surface area that is accessible to the ionic electrolyte. Similar cells in the past have used polyvinylidene fluoride, a conducting polymer, as a binder material. However, such cells were not able to long survive the enormous change in the volume of the sulfur layers during charge and discharge of the cell. To ameliorate this problem, the Berkeley team substituted an elastomeric co-polymer of styrene butadiene rubber and carboxymethyl cellulose for the binder.
The electrolyte was also changed in several ways from the traditional setup (and this being chemistry, you might need to brace yourself for some challenging terminology here). While the same electrolyte salt (lithium bis(trifluoromethanesulfonyl)imide) was used, the solvent was a mixture of nmethyl-(n-butyl) pyrrolidinium bis(trifluoromethanesulfonyl)-imide (PYR14TFSI), 1,3-dioxolane (DOL), dimethoxyethane (DME) with 1 M lithium bis-(trifluoromethylsulfonyl)imide (LiTFSI), and lithium nitrate (LiNO3).
This translates to combination which nicely balances the range of operating temperature, viscosity, and ionic conductivity required for efficient Li/S cell operation. The tendency of the cell to form lithium polysulfides was also reduced by the introduction of the DOL and DME fractions.
The lithium nitrate was added to reduce damage to the surface of the lithium metal anode, which had been observed to result from multiple charge/discharge cycling. A traditional coated plastic separator (high porosity polypropylene) was used to prevent the flow of electrons through the electrolyte while permitting free flow of the lithium ions.
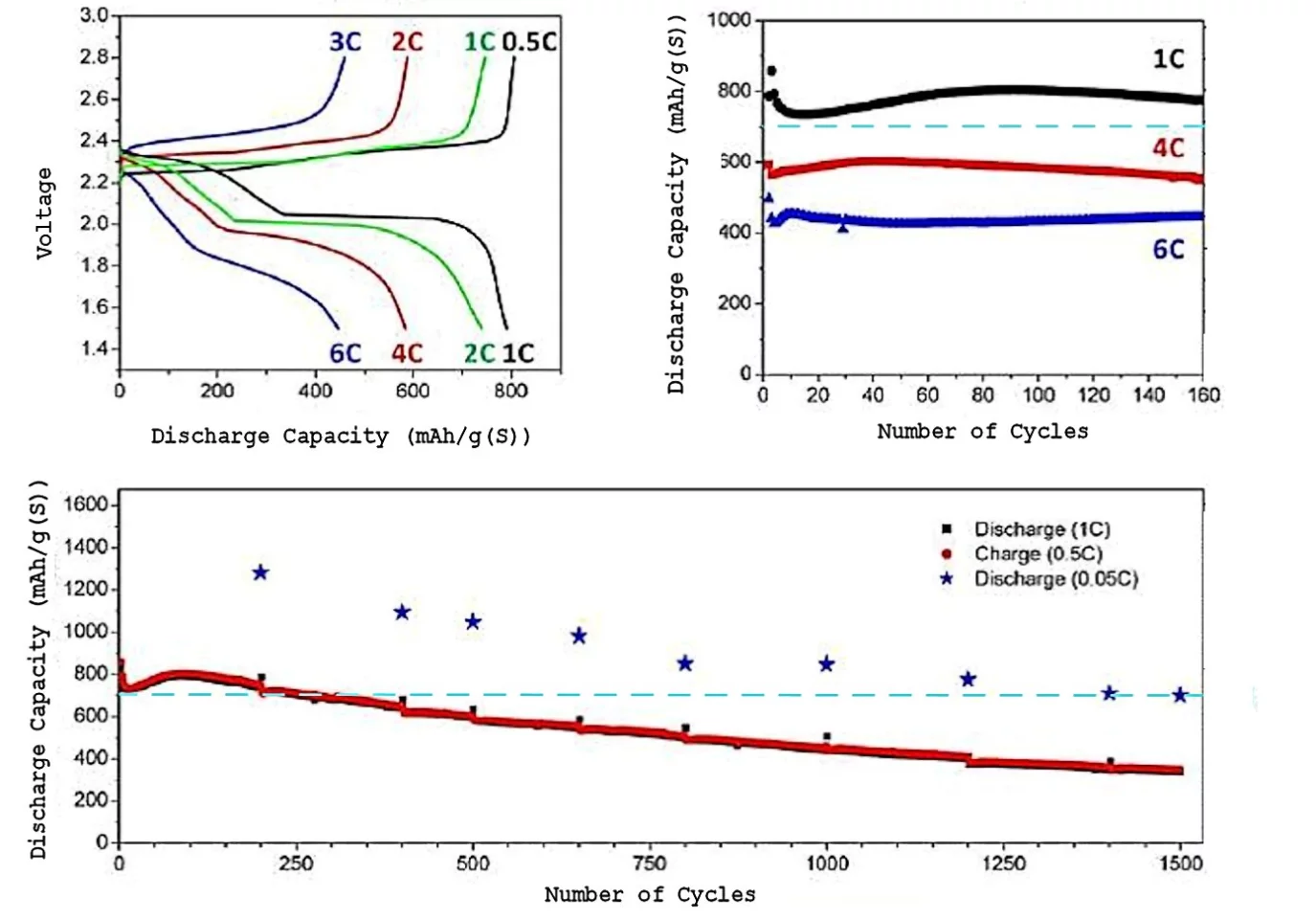
The result of these changes is greatly increased Li/S cell performance. When the Li/S cell was charged and discharged at a 20-hour rate (C=0.05), an initial specific energy of 500 Wh/kg (more than twice that of Li-ion batteries) was still providing as much energy capacity as a fresh Li-ion battery after 1500 charge/discharge cycles. When the charge/discharge rate is increased to a one-hour rate (C=1.0), the energy capacity decreases by a factor of about 40-50 percent, but the cell continues to function well past 1,500 cycles.
When the Li/S cells were operated at very large power output (C=6.0, meaning that a cell would charge or discharge in 10 minutes) even after 150 cycles the specific energy of the cell was larger than that of a fresh and pampered Li-ion cell. This capability for very large power charge and discharge was quite sensitive to the amount of lithium nitrate added to the electrolyte.
The potential price point for Li/S cells following the new design is potentially as low as US$100/kWh of storage capacity. Beyond making possible lithium/sulfur batteries with unprecedented specific energy, rate capacity, and long cycle life, many of the innovations made by the Berkeley team may also be useful in designing better and less expensive Li-ion cells. The Li/S cell for the first time has demonstrated its potential to challenge the dominant Li-ion battery chemistry in the big leagues of electric cars.