Exciting times are ahead in the high-tech industries with the discovery that a new class of materials mimic the special electronic properties of graphene in 3D. Three independent groups have found the same type of superfast massless charge carriers in bulk sodium bismuthide and cadmium arsenide as give graphene its unusually large electrical and thermal conductivity.
Graphene is a semimetal (zero-gap semiconductor), meaning that there are very few charge carriers compared to a normal metal. While semimetals generally have far smaller electrical and thermal conductivity than metals, this is not the case in graphene, which has an intrinsic resistivity about two-thirds of the resistivity of silver.
Why is graphene such a good electrical and thermal conductor? Electrical conduction in most materials is carried out by the motion of complex electronic excitations called quasiparticles, which result from the interaction of electrons with the graphene lattice. In normal materials, these quasiparticles act like electrons (or holes) which have an effective mass that, because of these interactions, can be larger or smaller than that of an electron, but otherwise behave much the same. Quasiparticles in these materials have parabolic energy, as shown in the left hand figure below.
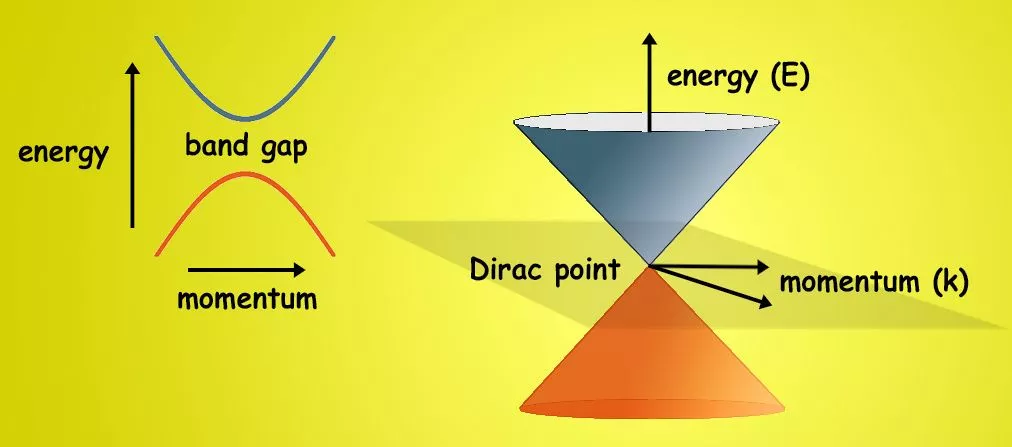
Something else happens in graphene. The quasiparticles which transport charge behave as if they had no mass; that is, their effective mass is zero. (The mass of the electrons hasn't vanished, but is hidden by the interactions between the graphene lattice and the electrons.)
This means that the speed of the quasiparticles through graphene is fixed at a roughly constant speed (depending on the angle of the Dirac cones shown in the right hand figure above) which turns out to be about 0.3 percent of the speed of light. The resulting properties are not those that would result from the effective charge carrier mass going to zero in a normal material. This highly unusual behavior gives graphene the special properties that make it so valuable as a foundation for electronic devices.
Three different groups have now, essentially simultaneously, discovered bulk materials in which massless quasiparticles carry charge through the materials in three dimensions – the 3D analog of 2D graphene. These materials, which include sodium bismuthide, or Na3Bi (discovered by researchers from the University of Oxford, SLAC National Accelerator Laboratory, Lawrence Berkeley National Laboratory, and Beijing National Laboratory) and cadmium arsenide, or Cd3As2 (discovered by researchers from IFW Dresden, Princeton University, and Technische Universitat Dresden, and independently by researchers from Princeton University, National Taiwan University, National Tsing Hua University, National University of Singapore, and Northeastern University) fall into a new class of materials called three-dimensional topological Dirac semimetals (3DTDS).
First contemplated in 1937, 3DTDS were formally predicted by theoretical models in 2011.
A 3DTDS is a bulk material in which conductivity is dominated by charge carriers (quasiparticles) which are massless in all three dimensions. Such charge carriers are called 3D Dirac fermions. Being massless, 3D Dirac fermions travel at a constant, typically quite high speed through the 3DTDS, resulting in unusually high conductivity, highly enhanced magnetoresistance, and properties well suited for efficient optical, pressure, and strain sensors.
All three groups were guided by theoretical predictions to examine the materials they chose. The tool of choice for studying the details of charge carriers is angle-resolved photoemission spectroscopy (ARPES). In this technique, very soft x-rays are directed at a surface, which cause electrons to be emitted from the excited surface at angles and speeds from which a detailed picture of the electronic band structure of the material being studied can be constructed.
In cadmium arsenide, the velocity of the 3D Dirac fermions was measured at approximately 1.6x10^6 m/s in all directions, or about 60 percent faster than the 2D Dirac fermions move through graphene. This is in good agreement with the experimental observation that the charge carrier mobility of Cd3As2 is very large, in fact of the same order of magnitude as that of graphene.
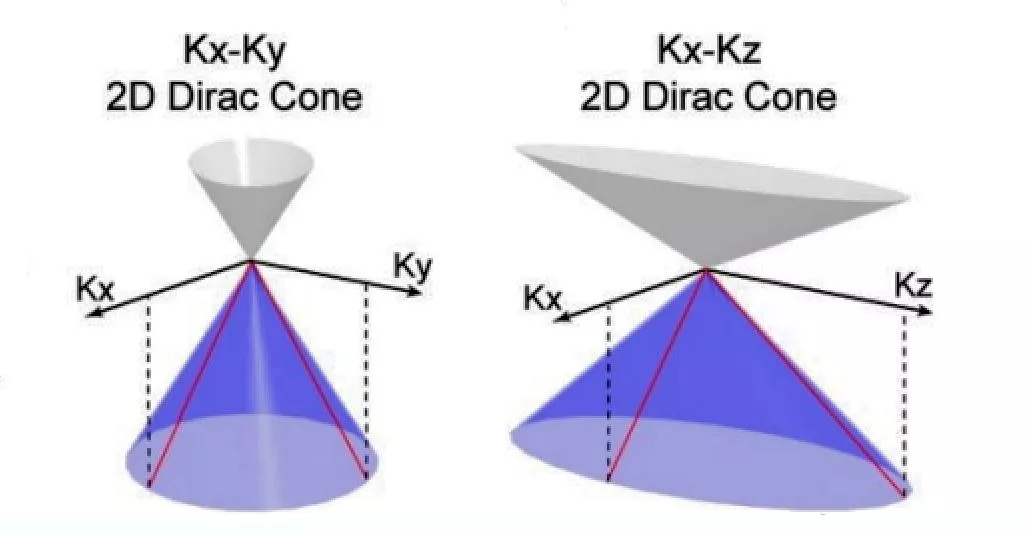
In sodium bismuthide, a layered compound, the velocity of the 3D Dirac fermions depends on the direction in which they travel through the material. In the plane of the layers, the speed is nearly the same in all directions at about 3.74x10^5 m/s, but perpendicular to the layers, the speed is 2.89x10^4 m/s. Thus, the Dirac cones shown above have different angles in different directions. Still, the conduction is by massless quasiparticles in all directions, so Na3Bi is still a 3DTDS.
So what does all this mean for applications? For a start, faster charge carriers means faster devices. In addition, the difficulty in preparing large high-quality graphene layers suggests that devices which are today considered for implementation in graphene might be easier to fabricate using bulk 3DTDS materials.
A very useful property of 3DTDS materials is their magnetoresistance. A material is magnetoresistive if its electrical resistance depends on the size of an applied magnetic field. In most materials, resistance changes as the square of the applied field, meaning that the change for small magnetic fields is very small indeed.
Magnetic field sensors based on the giant magnetoresistance effect are used in hard disk drives to sense the magnetically encoded data on the disk. In 3DTDS materials, however, that the charge carriers are massless leads to very large quantum magnetoresistance that depends linearly on the applied magnetic field. The result is that magnetic sensors based on 3DTDS materials will be far more sensitive to small changes in magnetic field. This property could lead to faster hard drives with more storage capacity as well as more sensitive magnetic sensors for other purposes.
Also expected is giant diamagnetism (the ability to expel a magnetic field), which should be much stronger than found in bismuth, the most highly diamagnetic bulk material currently known. Giant diamagnetism is primarily useful in magnetically levitated bearings at this point in time, but could open up a range of new applications. Other new properties make 3DTDS materials particularly favorable for applications in spintronics.
A question that often comes up involves superconductivity. Superconductors have essentially zero electrical resistance and exhibit the Meissner effect, in which magnetic field lines are completely pushed out of a superconductor (essentially perfect diamagnetism). As 3DTDS materials have massless charge carriers, very large conductivity, and have giant diamagnetism, is a 3DTDS something like a bad superconductor?
Tempting as the thought is that there is some relation between these apparently similar properties, superconductors and 3DTDS obtain their special properties in completely different ways. In superconductors, electrons in the material join together in Cooper pairs. A superconductor's special properties result from the formation of a superfluid of Cooper pairs, producing zero electrical resistance and the Meissner effect.
However, there is no electronic instability in 3DTDS, and nothing like a conducting superfluid forms. Massless charge carriers are usually associated with very small electrical resistance, but cannot produce zero resistance. In addition, while the giant diamagnetic susceptibility of a 3DTDS material may be as large as -0.001 or more, the perfect diamagnetism of a superconductor is associated with a diamagnetic susceptibility of -1. Tempting as the analogy may appear, it is not useful in practice. Topological superconductors have been predicted, but that is a story for another day.
Sources: arXiv (Princeton); arXiv (IFW Dresden) ;arXiv (Oxford)