Recently the media has been saturated with overly-hyped reports that NASA's Alpha Magnetic Spectrometer (AMS) may have detected dark matter. These claims may have some justification if the word "may" is shouted, but they rest on a number of really major assumptions and guesses, some of which are on weak and shifting soil. So just what was seen in the experiment, and what are the possible explanations?
Antimatter – setting the stage
Cars, trucks, planets, and other materials which we encounter in everyday life are composed of subatomic particles such as electrons, proton, and neutrons. However, another kind of matter known as antimatter was first predicted by Paul Dirac in 1931, followed quickly by Carl Anderson's discovery in 1932 of the antielectron, or positron, in cosmic rays.Each of the subatomic particles we know has an antiparticle, which have the same mass as their corresponding subatomic particles, but with the opposite charge. When a particle and its antiparticle collide, they are converted into gamma rays, often combined with lighter particle-antiparticle pairs. However, isolated antimatter looks and behaves identically to normal matter. (So far, anyway. There are some hints from experimental data that there may be some minor differences, but the jury is still out.)
How is antimatter detected? Normally, this is done by looking for gamma rays produced when antimatter is annihilated on contact with normal matter, or by measuring its properties as it moves through matter.
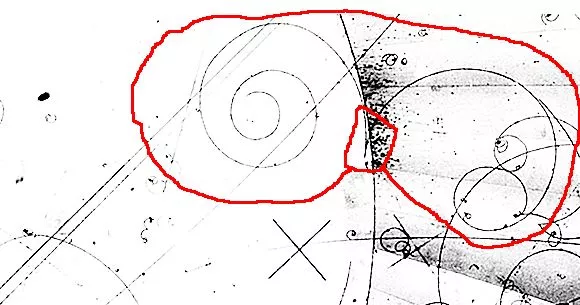
For example, in a cloud chamber or a bubble chamber, a particle moving through the detector leaves a track of its motion by ionizing the matter in the chamber. If you apply an electric or a magnetic field properly, an electron moving to the right will curve up, while a positron moving to the right will curve down. The difference reflects their opposite charges. More sophisticated detectors can measure the mass, charge, energy, and direction of a particle or an antiparticle.
Antimatter in the Universe
Both matter and antimatter were created during the early moments of the Big Bang, but it's believed that the antimatter currently in the Universe is not left over from this event. This is because matter and antimatter appear to have mutually annihilated in the high-density environment about a minute after the Big Bang, leaving one part of normal matter from a billion parts of the original matter-antimatter mix. No one currently knows why the early Universe showed a preference for matter at the cost of antimatter (this is one of the hints mentioned earlier), but here we are.
These days, antimatter is created everywhere in the Universe that is sufficiently hot or where high-energy particle collisions take place. Supernovae, pulsars, neutron stars, black hole accretion discs, and quasars – even planetary thunderstorms are known to be sources of antimatter.
The main source of cosmic positrons is the interaction of high energy cosmic rays colliding with interstellar gas and dust. These are called secondary positrons, because they are generated as a product of the primary cosmic ray collisions. Those we see are typically produced within a few thousand light years of our Solar System, as positrons from more distant sources lose energy and eventually are trapped before they can reach Earth.
This source of electron-positron pairs peters out for positrons with energy over a few GeV. The balance works out so the the ratio known as the positron fraction (the number of positrons detected divided by the total number of electrons and positrons detected at that same energy) decreases steadily for positron energy more than about one GeV.
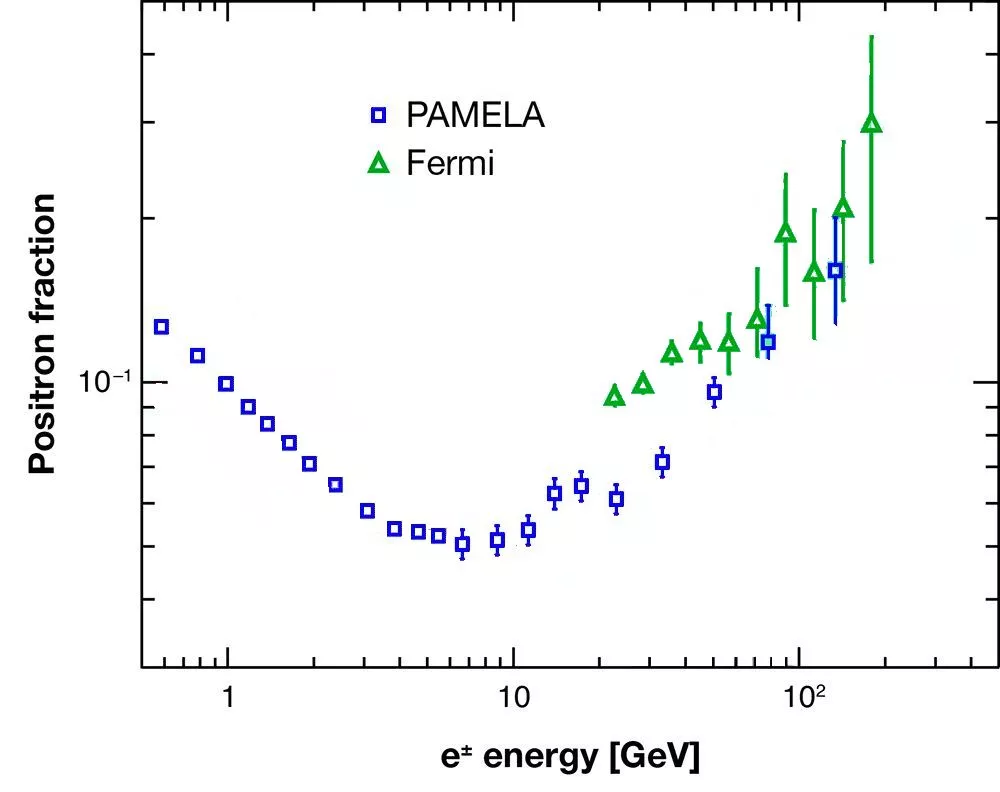
Experimental studies of the positron fraction in either the upper atmosphere or in space have been carried out since the 1960s. These have suggested that the decrease of the positron fraction with increasing energy slows beginning at a few GeV, and then the positron fraction roughly doubles as the energy approaches 100 GeV. At this point the positron fraction is over ten percent, which is quite a lot of antimatter, suggesting that another source of high energy positrons must be active in our galactic neighborhood.
The Alpha Magnetic Spectrometer (AMS)
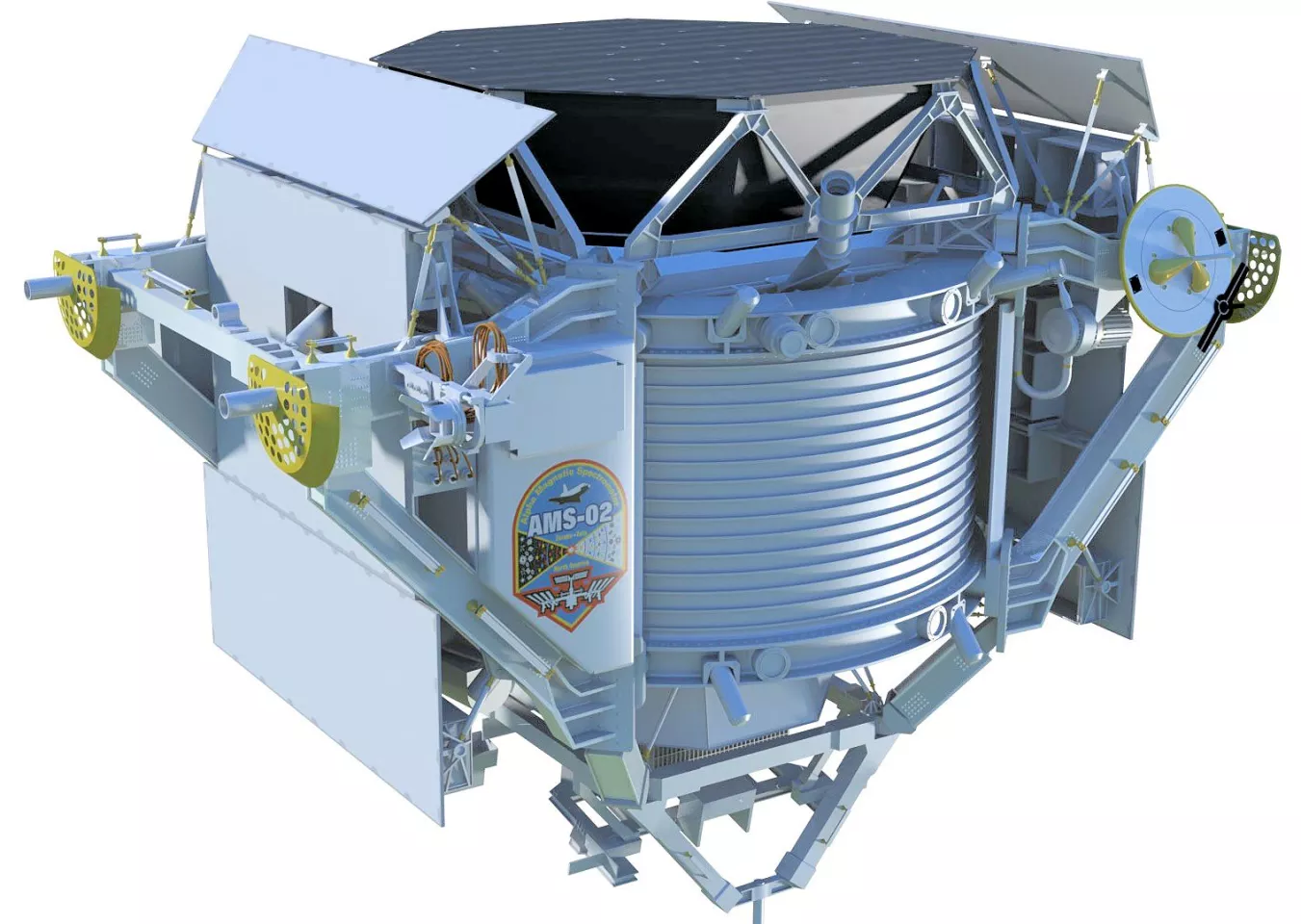
The Alpha Magnetic Spectrometer (AMS) got its original impetus from American politics. In 1993, the U.S. Congress cancelled construction of the Superconducting Supercollider (SSC), which was to have a design energy of 20 TeV (producing 40 TeV proton-proton collisions). This cancellation left a very large tunnel in Texas (now being used to house a data center), and dramatically reduced the opportunities for discovering new particles and new physics until the 2010 advent of the substantially less powerful LHC.
Partially in response to the SSC cancellation, Nobel Laureate Prof. Samuel Ting of the MIT Department of Physics proposed in 1995 that NASA construct and launch the space based Alpha Magnetic Spectrometer. The AMS is in essence a very capable particle detector whose purpose is to investigate extreme matter in the universe by carefully measuring the properties of very high energy cosmic rays. To do so, it is designed to discriminate particles from electrons to nuclei at energies up to 2,000 GeV.
In the end, NASA agreed to give the AMS space on the ISS and to transport it via the Space Shuttle, but Prof. Ting had to construct it under the auspices of the US Department of Energy. He funded this effort by convincing the DOE to take on the project, which also attracted a good deal of support from other countries. A simple version of the detector was sent to orbit for a few days on the Space Shuttle in 1998, and the full AMS was launched and installed on the ISS in May of 2011.
As an aside, in a pattern not unusual for space projects, the initial AMS estimated cost of US$33 million with a launch date in 2003 ballooned to an instrument cost estimated at US$1.5 billion and a launch in 2011 that required the US Congress to approve an additional flight at the end of the Space Shuttle program. It appears that even minds capable of elucidating the fundamental nature of the universe cannot accurately predict program costs.
The AMS positron fraction data
The AMS has recorded a total of 25 billion cosmic ray interactions in the past 18 months, of which nearly seven million involved electrons and positrons having energies between 0.5 and 350 GeV. Analysis indicates that the positrons appear to arrive equally from all directions. Despite the large number of measurements, at energies larger than 250 GeV there are not yet sufficient particles detected on which to produce trustworthy data.
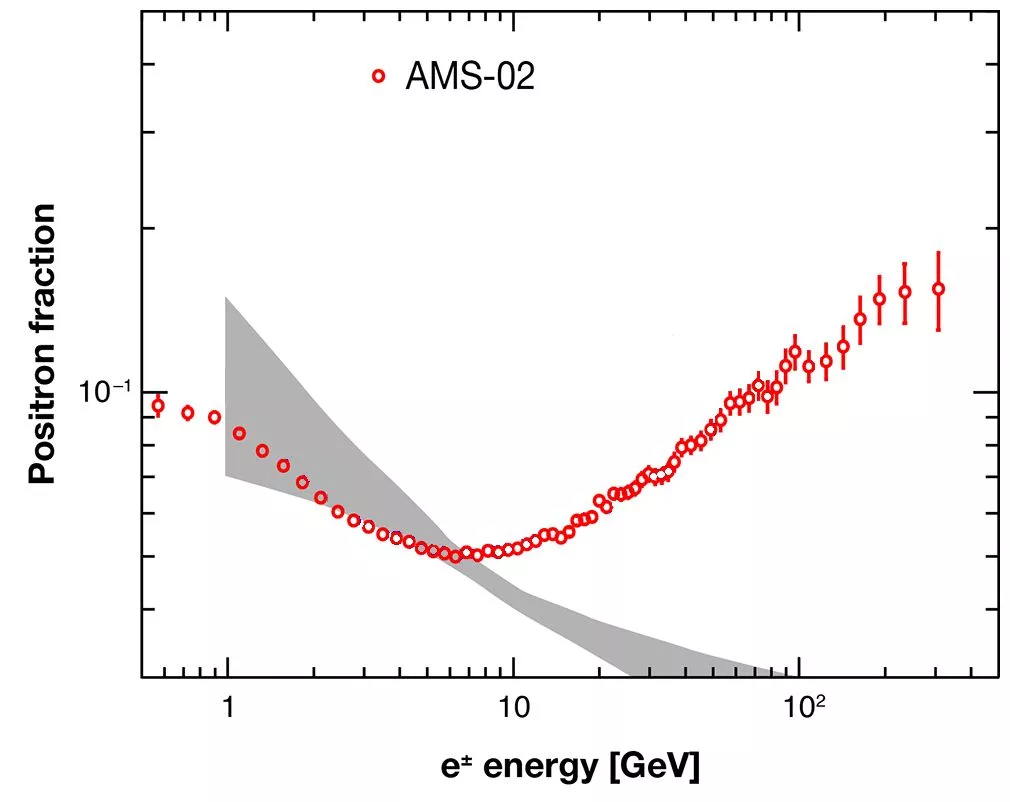
In essence, although the new data is more precise, at this point the impact is mainly limited to confirming the increase in positron fraction seen in previous studies. The one hint of new behavior is that the increase in positron fraction may be slowing as energies over 200 GeV are examined.
The possible connection to dark matter
There are numerous possible explanations for the large number of positrons at high energies, but they narrow to three main classes. The first is that they are due to local astrophysical sources, such as pulsars in our general neighborhood. There are fairly realistic sounding mechanisms by which pulsars could generate high energy electrons and positrons, and such particles could be smeared out by the influence of interstellar electric and magnetic fields to appear to come from all directions equally. However, it seems a rather large coincidence that the locations of the pulsars and the configuration of interstellar electromagnetic fields combine to make it appear that the high energy positrons come from all directions. Such sources of high energy positrons are taken seriously, but many astronomers remain skeptical.
The second explanation is that they are due to dark matter annihilations and/or decays. The third is that they are generated by something else, from a normal astrophysical source that just hasn't yet been considered to something entirely new and unexpected. Of these three, the most compelling is arguably the second, so let's take a look at how realistic it is.
Our latest cosmological models indicate that a full 27 percent of the mass-energy of the Universe is in the form of cold dark matter. Dark matter is thought to be composed of particles that have considerable mass, but only interact with "normal matter" through gravitation, and perhaps the weak nuclear force. As a result, while these particles contribute to the mass and pressure of the Universe, we can't directly see them.
So what might dark matter actually consist of? That such dark matter particles could exist isn't a big stretch. The extraordinarily elusive neutrinos would qualify as dark matter, as they only have weak and gravitational interactions with the rest of the Universe, but they don't have enough mass.
The current hot idea is that dark matter consists (at least in part) of hypothetical particles called WIMPS (Weakly Interacting Massive ParticleS), and that mutual annihilation of WIMPS is the source of the high energy positrons.
However, the Standard Model of Particle Physics, which rather nicely explains every known particle, contains no possible WIMP candidates. If the positron excess is due to WIMP annihilation, then new physics is required at a fundamental level.
The reason this explanation is dicey, but very attractive at the same time, is that it depends rather strongly on at least two paradigm-altering assumptions – supersymmetry and the existence of Majorana fermions.
Supersymmetry, in which a new symmetry is postulated between fermions and bosons, so that if you turn all fermions into bosons (and vice versa), the physics of the system doesn't change. However, this doesn't hold with current observations of the Universe, so theorists further postulate that the symmetry is broken, meaning that it is only exact at very large energies.
Supersymmetry produces new aspects of particle physics that are so alluring that it is sometimes taken for granted, even though there is as yet no experimental data suggesting that supersymmetry actually holds in the universe. However, supersymmetric versions of the Standard Model do contain excellent candidates for dark matter WIMP particles.
The lead candidate for a supersymmetric WIMP is a neutralino, which is not only undiscovered, but is predicted to be a Majorana fermion, a particle which is its own antiparticle. Although collective excitations in superconductors that behave as Majorana particles have been seen, there is no evidence as yet for fundamental Majorana particles.
If supersymmetry is how the Universe works, and neutralinos indeed have the predicted properties, then although an individual neutralino may be stable, a pair can mutually annihilate when they interact. Such interactions would be rare, owing to the large distances between neutralinos and their very small interaction cross-sections. However, if neutralino masses are, as suspected, in the general range of one TeV, there is plenty of energy in an annihilation to make gamma rays and normal matter particle-antiparticle pairs.
It has also been suggested that the dark matter side of the Universe may be as complex and diverse as is the part to which we are accustomed. If true, this would open the possibility of dark matter annihilations, reactions, and decays which might still produce electrons and positrons without requiring any Majorana particles.
Can AMS data really add up to dark matter?
According to the initial CERN report, the results of the AMS data and analysis, "are consistent with the positrons originating from the annihilation of dark matter particles in space, but not yet sufficiently conclusive to rule out other explanations." However, early media coverage was strongly oriented toward explaining the observed positron excess as associated with dark matter annihilation, and appeared dismissive of other explanations. Following a discussion of dark matter annihilation as the source of the positron excess, the report also states that their model fits the data "surprisingly well" – without mentioning that the model is not connected with dark matter in any sense. As a result, the subsequent media reports often read as if dark matter had indeed been discovered, and all that remained was to dot the i's and cross the t's. The result was to spread an incorrect impression of the science involved far and wide.
So, what factors argue against the dark matter hypothesis? Probably the clearest signature (although not proof) that the positron excess is the result of dark matter annihilations would be if the positron excess declines abruptly above a given energy. This would suggest that the source of the positrons has a maximum energy, likely associated with the mass of the dark energy particles. If such a maximum energy is seen at a realistic WIMP mass, that will be evidence for, but not proof of, dark matter annihilation. In the present absence of this signature, there is very little in the data pointing toward the dark matter explanation.
Another difficulty is that, all things being equal, one would expect nearly as many proton-antiproton pairs to be generated in WIMP annihilation as electron-positron pairs, as there is plenty of energy to go around. In fact, though, the AMS data shows that essentially no antiprotons are detected in the energy range where the positron excess is observed. This anomaly would likely require yet another bit of postulated new physics to explain. One hates to add unrelated new physics to fix an explanation already based on new physics.
A final point is that most dark matter appears to be located in the galactic halos, or in filaments connecting galaxies and galactic clusters, rather than amongst the stars in the spiral arms. If the dark matter annihilation mechanism for the positron excess is correct, then there are also a large density of extremely high energy electrons and positrons in intergalactic space. As a result, the extragalactic gamma ray spectrum would show the effects of scattering from these energetic charged particles. Specifically, the average gamma ray energy would be increased in a specific manner by the inverse Compton effect. Such an effect is not observed, which strongly constrains the electron-positron density, and along with it the density of dark matter, in the galactic halo.
It would seem to require a perfect storm of just the right new physics, plus some complex explaining away of known physics, for the dark matter annihilation hypothesis for the positron excess to be true. Stranger things have happened in scientific history, of course. The proof of the pudding, however, comes when such ideas are tested in many more-or-less unrelated ways – the final result is usually a simple, nearly inevitable explanation. The current and future AMS data alone cannot bring astrophysics to that level concerning the source of excess high-energy cosmic positrons and, given the difficulties inherent in other experimental directions, the issue of dark matter is not likely to be resolved soon.
Prof. Jonathan Feng of UC Irvine sums the situation up nicely: "If anything mentioned here is correct, life will be very interesting in the coming years!"
Source: AMS-02